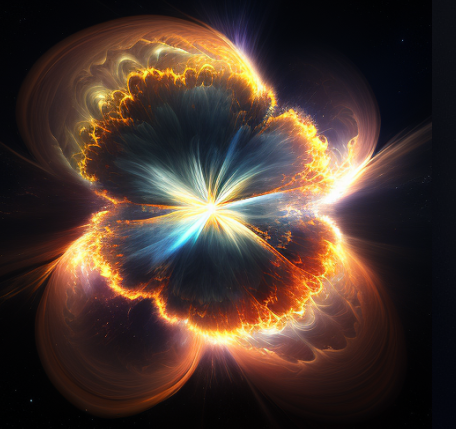
Chapter 2: Galaxies
Contents
1. Galaxy Evolution
-
Dark matter halos form: Galaxies are thought to form within large-scale structures called dark matter halos. These halos are made up of invisible dark matter that exerts a gravitational force, attracting gas and other matter.
-
Gas and dust accumulation: As gas and other matter fall into the dark matter halo, they accumulate at the center of the halo, forming a rotating disk or sphere.
-
Star formation: As the gas and dust within the rotating disk or sphere continue to accumulate, they begin to collapse under the force of gravity, leading to the formation of new stars.
-
Galaxy mergers: Over time, smaller galaxies and gas clouds may merge with the growing galaxy, contributing additional material and leading to the formation of more complex structures such as spiral arms, bars, and bulges.
-
Ongoing evolution: Once a galaxy has formed, it continues to evolve and change over time, as stars are born and die, and the galaxy interacts with its environment.
2. Galaxy Types
Galaxies come in various shapes and sizes, ranging from elliptical, spiral, to irregular. The shape of a galaxy is determined by many factors, including its age, mass, and interactions with other galaxies.
-
Galaxies are classified into three main types: spiral, elliptical, and irregular.
-
Spiral galaxies have a mix of old and young stars, with younger, bluer stars concentrated in the galaxy's arms and older, redder stars found in the central bulge.
Spiral galaxies also contain large amounts of gas and dust, which can give rise to ongoing star formation.
-
Elliptical galaxies, on the other hand, have a more spherical shape and are made up of older stars with little or no gas and dust.
Elliptical galaxies are generally older and contain more old, red stars than younger, blue stars.
Elliptical galaxies have a more spherical shape and have little gas or dust. The stars in elliptical galaxies tend to be more evenly distributed throughout the galaxy, with less distinct structure than the stars in spiral or irregular galaxies.
Messier 87 (M87), which is located in the constellation Virgo and is one of the largest and most massive galaxies in the nearby universe. M87 has an elliptical shape and lacks the flattened, rotating disk structure of spiral galaxies like the Milky Way.
-
Irregular galaxies have a mix of old and young stars, with ongoing star formation taking place in regions of gas and dust throughout the galaxy. Irregular galaxies often have complex and irregular structures, with stars and gas distributed in a more haphazard way than in spiral or elliptical galaxies.
3. Star Formation
Star formation is a fundamental process that takes place within galaxies, where clouds of gas and dust coalesce and collapse under their own gravity, forming new stars. The process of star formation is complex and can vary depending on the type of galaxy and its environment. Presently, there are somewhere between one and four hundred billion stars in the Milky Way. From the outside, the Sun would look very average.
-
In spiral galaxies, the gas and dust are concentrated in the arms of the galaxy, where the gravitational forces and shocks from the spiral arms trigger the formation of new stars. These stars are typically young and massive, and they generate intense radiation that heats and ionizes the surrounding gas, creating colorful nebulae that are visible to telescopes.
-
In elliptical galaxies, star formation is less active, as the gas and dust have been largely depleted over time. However, some elliptical galaxies do contain pockets of gas and dust that can still form new stars.
Stars evolve over time through a sequence of stages, starting from the fusion of hydrogen atoms into helium in their cores, to the production of heavier elements through nuclear fusion in their cores and shells. The exact path of evolution depends on the mass of the star, with more massive stars evolving faster and through different stages than less massive stars. Eventually, all stars will exhaust their fuel and undergo a final phase of evolution that results in the formation of a white dwarf, neutron star, or black hole, depending on their mass.
The Hertzsprung-Russell (HR) diagram above is a graphical representation of the relationship between a star's surface temperature and its luminosity. It shows that stars of different spectral types have different luminosities, and that stars with higher surface temperatures are generally more luminous than cooler stars.
-
-
Mass: The mass of a star is one of the most important factors in determining its properties and evolution. More massive stars are generally larger, hotter, and more luminous than less massive stars, because they have more material to burn and produce more energy. Conversely, low-mass stars are smaller and cooler than high-mass stars, because they burn their fuel more slowly and produce less energy.
-
Temperature: The temperature of a star refers to the average kinetic energy of the gas particles in its outer layers, and is usually expressed in units of Kelvin (K). Hotter stars have higher temperatures than cooler stars, and this affects their color and spectral characteristics. For example, the hottest stars appear blue-white, while the coolest stars appear red.
-
Luminosity: The luminosity of a star refers to the total amount of energy it emits per unit time, and is usually expressed in units of solar luminosities (L☉). Luminosity is determined by the star's size, temperature, and fuel consumption rate, and is one of the primary factors that determines a star's brightness and visibility.
-
Age: The age of a star refers to the length of time it has been burning hydrogen in its core, and is usually expressed in units of billions of years. A star's age is determined by its mass and chemical composition, and affects its properties and evolution. For example, young stars tend to be larger and hotter than old stars, because they are still burning hydrogen rapidly in their cores.
-
Main sequence stars are the most common type of star in the universe and are defined by a stable phase of their life cycle where they fuse hydrogen into helium in their cores. The mass of a main sequence star determines its size, temperature, and luminosity, with more massive stars being hotter, larger, and more luminous than less massive stars.
The relationship between a star's luminosity and temperature creates a distinct line on a graph known as the Hertzsprung-Russell (HR) diagram. The majority of main sequence stars fall along a narrow band on the HR diagram known as the "main sequence," where their luminosity increases with temperature.
As a main sequence star burns through the hydrogen fuel in its core, it will eventually evolve into a different phase of its life cycle. This transition is marked by a departure from the main sequence on the HR diagram, as the star expands and becomes a red giant or supergiant, depending on its mass.
In addition to the internal factors that influence star formation, galaxies can also be influenced by external factors such as interactions with other galaxies or the surrounding intergalactic medium. For example, when galaxies collide, the shock waves generated can trigger intense bursts of star formation. In contrast, when a galaxy is stripped of its gas by interactions with other galaxies or the intergalactic medium, its star formation can be dramatically reduced or even halted altogether.
4. Milky Way Collision
About thirteen billion years ago in our region of the universe, stars began forming in two distinct stellar systems. One system was a dwarf galaxy that we now refer to as Gaia-Enceladus, while the other was the precursor to the Milky Way. It was around four times larger in mass and had a greater proportion of heavy elements than the dwarf galaxy. Roughly 11.5 billion years ago, a violent collision occurred between these two systems, resulting in some of their stars being set into chaotic motion and eventually forming the halo of the present-day Milky Way. Following this, there were intense periods of star formation until about 6 billion years ago, at which point the gas settled into the galaxy's disk and formed the thin disk that we observe today.
-
Researchers tracked the history of a prominent star called "nu Indi" located in the Indus constellation, visible from the southern hemisphere of Earth. According to the scientists, nu Indi was present within the Milky Way prior to the merger with the Gaia-Enceladus structure, and its orbit around the center of the galaxy was changed by the merger. The star's orbit served as an indicator for when the collision occurred since stars, like planets, also have orbits.
5. More Elements From Bigger Stars
We saw last chapter that he lightest elements, Hydrogen and Helium were produced in two ways: one just after the Big Bang through primordial nucleosynthesis and the other through stellar nucleosynthesis called Carbon burning in low mass stars.
The stages of stellar nucleosynthesis depend on the mass of the star, with low-mass stars fusing hydrogen and helium into carbon and oxygen, intermediate-mass stars fusing carbon and oxygen into heavier elements like neon and magnesium, and high-mass stars fusing neon, magnesium, and silicon into elements like iron, nickel, and chromium before undergoing a supernova explosion.
The elements produced by stellar nucleosynthesis are recycled into the interstellar medium, where they can be incorporated into new stars and planets. Over time, the abundance of heavy elements in the universe has increased due to the continued production of these elements in stars and supernova explosions.
-
-
Low-mass stars (less than about 8 solar masses): These stars undergo hydrogen burning in their cores, where hydrogen is fused into helium. As the hydrogen in the core is exhausted, the star begins to fuse helium into heavier elements like carbon and oxygen in a process known as helium burning. Low-mass stars never become hot enough to fuse elements heavier than carbon and oxygen, so they end their lives as white dwarfs composed mostly of carbon and oxygen.
-
Intermediate-mass stars (between 8 and 10 solar masses): These stars undergo the same stages of nuclear burning as low-mass stars, but they can reach higher temperatures and pressures in their cores. This allows them to fuse carbon and oxygen into heavier elements like neon, magnesium, and silicon. Intermediate-mass stars eventually become white dwarfs composed of a variety of elements, depending on their mass.
-
High-mass stars (greater than 10 solar masses): These stars undergo all of the same stages of nuclear burning as intermediate-mass stars, but they can also reach even higher temperatures and pressures in their cores. This allows them to fuse neon, magnesium, and silicon into heavier elements like iron, nickel, and chromium in a process known as silicon burning. High-mass stars eventually undergo a supernova explosion, which produces elements heavier than iron through a process known as explosive nucleosynthesis.
-
-
The carbon burning process is a nuclear fusion process that occurs in the cores of massive stars that have exhausted the hydrogen and helium in their cores. It occurs at temperatures of around 600 million degrees Kelvin and involves the fusion of carbon-12 nuclei to form heavier elements such as oxygen-16 and neon-20.
The carbon burning process is the second stage in a series of nuclear reactions that takes place in massive stars, after the initial fusion of hydrogen and helium into carbon and oxygen. It is a very rapid process, lasting only a few years in a massive star's lifetime, but it is responsible for producing a significant fraction of the elements heavier than helium in the universe.
-
Neon burning is a nuclear fusion process that takes place in the cores of massive stars that have exhausted the hydrogen and helium in their cores. It occurs at temperatures of around 1.5 billion degrees Kelvin and involves the fusion of neon-20 nuclei to form heavier elements such as magnesium-24, sodium-23, and oxygen-16.
The neon burning process is the third stage in a series of nuclear reactions that takes place in massive stars, after the carbon burning and helium fusion processes. It is a very rapid process, lasting only a few years in a massive star's lifetime, but it is responsible for producing a significant fraction of the elements heavier than helium in the universe.
The neon burning process requires high temperatures and densities to occur, and it produces a large amount of energy that is responsible for maintaining the pressure and temperature in the star's core. The fusion of neon into heavier elements is an essential step in the production of elements like silicon, sulfur, and iron, which are synthesized in later stages of a massive star's life.
-
Silicon burning is a nuclear fusion process that takes place in the cores of massive stars that have exhausted the carbon and oxygen in their cores. It occurs at temperatures of around 3.5 billion degrees Kelvin and involves the fusion of silicon-28 nuclei to form heavier elements such as iron-56, nickel-56, and chromium-56.
The silicon burning process is the last stage in a series of nuclear reactions that takes place in massive stars, after the initial fusion of hydrogen and helium into heavier elements like carbon and oxygen, and the fusion of carbon and oxygen into heavier elements like neon and magnesium. It is a very rapid process, lasting only a few days in a massive star's lifetime, but it is responsible for producing a significant fraction of the elements heavier than iron in the universe.
-
After silicon burning, no further energy can be extracted from the core of a massive star through nuclear fusion. At this point, the core of the star consists mainly of iron, which is the most stable element with the highest binding energy per nucleon. Since it is energetically unfavorable to fuse iron or any other element heavier than iron, the star's core can no longer generate enough energy to counteract the force of gravity.
This leads to the core collapsing under its own weight, which triggers a runaway fusion reaction that creates a shock wave that propagates outward through the star's envelope. This shock wave disrupts the star's structure and leads to a supernova explosion, in which the outer layers of the star are expelled into space at high speeds. This explosion produces a wide range of elements heavier than iron through a process known as explosive nucleosynthesis.
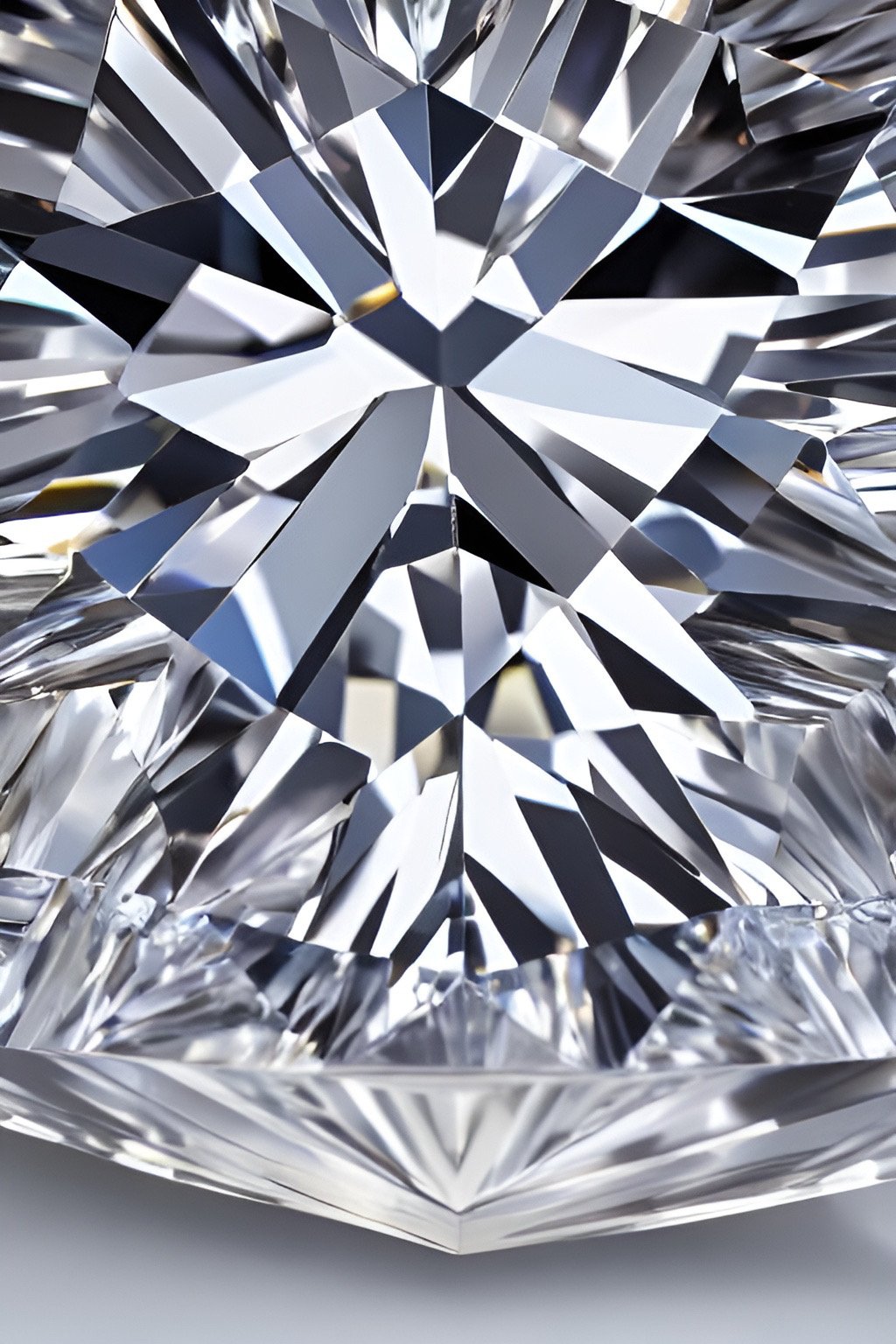
6. Minerals
What is a Mineral?
A mineral is a naturally occurring, inorganic solid substance that has a specific chemical composition and crystal lattice. There is ongoing debate among mineralogists about the precise definition of a mineral. Some researchers have proposed broader definitions that would include synthetic materials like glassy carbon and graphene, along with the inclusion of non-crystalline materials, such as volcanic glass, amber, and coal, which currently aren’t counted as minerals.
-
Chemical Composition refers to the specific types and amounts of chemical elements that are present in the mineral. For example, the mineral calcite is composed of calcium, carbon, and oxygen, with the chemical formula CaCO3. Not included in the chemical formula, many minerals contain small amounts of impurities or trace elements that are not part of the primary chemical composition of the mineral. These impurities can affect the color, texture, and other physical properties of the mineral, and can also provide important information about the geologic processes that formed the mineral.
-
Crystal Lattice is the three-dimensional arrangement of atoms, ions, or molecules bonded together in various ways to make up a mineral. The crystal lattice determines the physical and chemical properties of the crystal, including its shape, hardness, melting point, and electrical conductivity. Each atom, ion, or molecule is located at a specific position within the lattice, and these positions are determined by electrostatic, magnetic, or other types of interactions. The crystal lattice can be described by a unit cell, which is the smallest repeating unit of the lattice. The unit cell contains one or more atoms or ions, and it is repeated in all three dimensions.
-
Allotropes are different forms of similar minerals that have the same chemical composition but, distinct crystal structures or molecular arrangements. The variation of the crystal lattice results in unique physical and chemical properties.
Carbon is a mineral that has several well-known allotropes, including graphite and diamond. Graphite has a layered structure that gives it a soft, slippery texture, making it useful as a lubricant and in pencils. Diamond, on the other hand, has a tightly packed crystal structure that makes it the hardest known material, with uses in jewelry and cutting tools.
-
Mineralogists are debating how to improve the mineral classification scheme based on the formation mechanism of minerals. For example, all diamonds have the same basic chemical composition and crystal lattice, but diamond formed in a low pressure stellar atmosphere (AGB diamond) is much different than diamond formed by shock alteration of carbon-rich material (impact diamonds) and those crystallized at high pressure and temperature deep within the earth (Type 1 diamond)
What was the first mineral in the universe?
Given the initial high temperature, the first generation of stars were predominantly hydrogen and helium in gas form with no crystals. However, once larger mass stars started the process of carbon burning, elements like carbon, oxygen, silicon, and magnesium were produced. Carbon is the first element which tends to form a solid when bonded to itself making it capable of forming minerals.
Diamond, is the most likely candidate for the first mineral, created from pure carbon condensed in the expanding atmospheres of energetic stars. These diamonds were likely very small, considered nanodiamonds. Examples found in pre-solar meteorites have grain sizes ranging from a few to tens of nanometers in diameter, and contain just a small number of atoms.
Graphite, an allotrope of diamond likely formed next along with two other carbon bearing minerals, cohenite and moissanite. Nano-particles of titanium, iron, molybdenum, and zirconium carbides with high crystallization temperatures, almost as high as diamond, would have readily formed in the zones of an exploding star where carbon mixed with silicon or iron.
Building Minerals in Virtual Reality
-
The images above and materials described below are all made of carbon, but have different crystal structures resulting from different formation mechanisms. Not all are considered “minerals” because some do not have a regular crystal structure (like amorphous and glassy carbon) and others do not exist naturally (carbon nanotubes and graphene).
-
Diamond - Diamond is a crystalline form of carbon that has a three-dimensional lattice structure, with each carbon atom bonded to four neighboring carbon atoms. Diamonds are renowned for their extreme hardness and optical properties, and are used in jewelry, cutting tools, and industrial abrasives.
-
Graphite - Graphite is a soft, black form of carbon that consists of layers of carbon atoms arranged in a two-dimensional lattice structure. Graphite is used in pencils, lubricants, and as a conductor of heat and electricity.
-
Fullerene - Fullerenes are a class of allotropes of carbon that are composed of interconnected carbon atoms arranged in hexagonal and pentagonal rings. They are typically spherical or ellipsoidal in shape and are used in a variety of applications, including drug delivery and cancer treatment.
The most common fullerene is C60, which has a spherical shape and is composed of 60 carbon atoms. Other fullerenes, such as C70 and C84, contain 70 and 84 carbon atoms, respectively, arranged in different shapes and structures. In general, fullerenes can contain anywhere from a few dozen to a few hundred carbon atoms, depending on their specific structure and size.
-
Amorphous carbon - Amorphous carbon is a non-crystalline form of carbon that does not have a defined lattice structure. It is used in a variety of applications, including inks, coatings, and water purification.
Recent spectroscopic evidence indicates that carbonaceous grains are predominantly made of amorphous carbon (Mennella et al. 1998; Henning and Mutschke 2004).
-
Carbon nanotubes - Carbon nanotubes are cylindrical tubes of carbon atoms that have unique physical and electrical properties. They are used in electronics, materials science, and other high-tech applications.
-
Glassy carbon - Glassy carbon is a non-graphitizing form of carbon that is produced by pyrolysis of a phenolic resin. It has a glass-like appearance and is characterized by its high temperature resistance, chemical inertness, and low thermal expansion. Glassy carbon is used in a variety of high-temperature and corrosive environments, including in electrodes, crucibles, and high-temperature furnace components.
-
Graphene - Graphene is a single layer of carbon atoms arranged in a hexagonal lattice structure. It is one of the thinnest materials known and is characterized by its exceptional mechanical, electrical, and thermal properties. Graphene is a promising material for use in electronics, energy storage, and other high-tech applications, and is the focus of intensive research worldwide.
Quiz Time
Our Black Hole
Sagittarius A (Sgr A) is a supermassive black hole located at the center of the Milky Way galaxy. It is located about 25,000 light-years from Earth in the direction of the constellation Sagittarius, which is how it gets its name.
The mass of Sagittarius A is estimated to be about 4 million times that of the Sun, making it one of the most massive known black holes in the universe. Despite its immense size, Sagittarius A is not visible to the naked eye, as it is located behind a dense veil of gas and dust in the galactic center.
The presence of Sagittarius A was first detected in the 1970s through radio observations of the galactic center. Since then, numerous studies have been conducted to better understand the properties of this supermassive black hole. In recent years, astronomers have been able to observe the behavior of stars orbiting Sagittarius A, which has allowed them to make more precise measurements of its mass and other properties.
Sagittarius A is also believed to be the source of powerful radio and X-ray emissions, which are thought to be produced by gas and dust falling into the black hole's event horizon. Studying these emissions has provided important insights into the behavior of matter and energy in the extreme environments surrounding black holes.