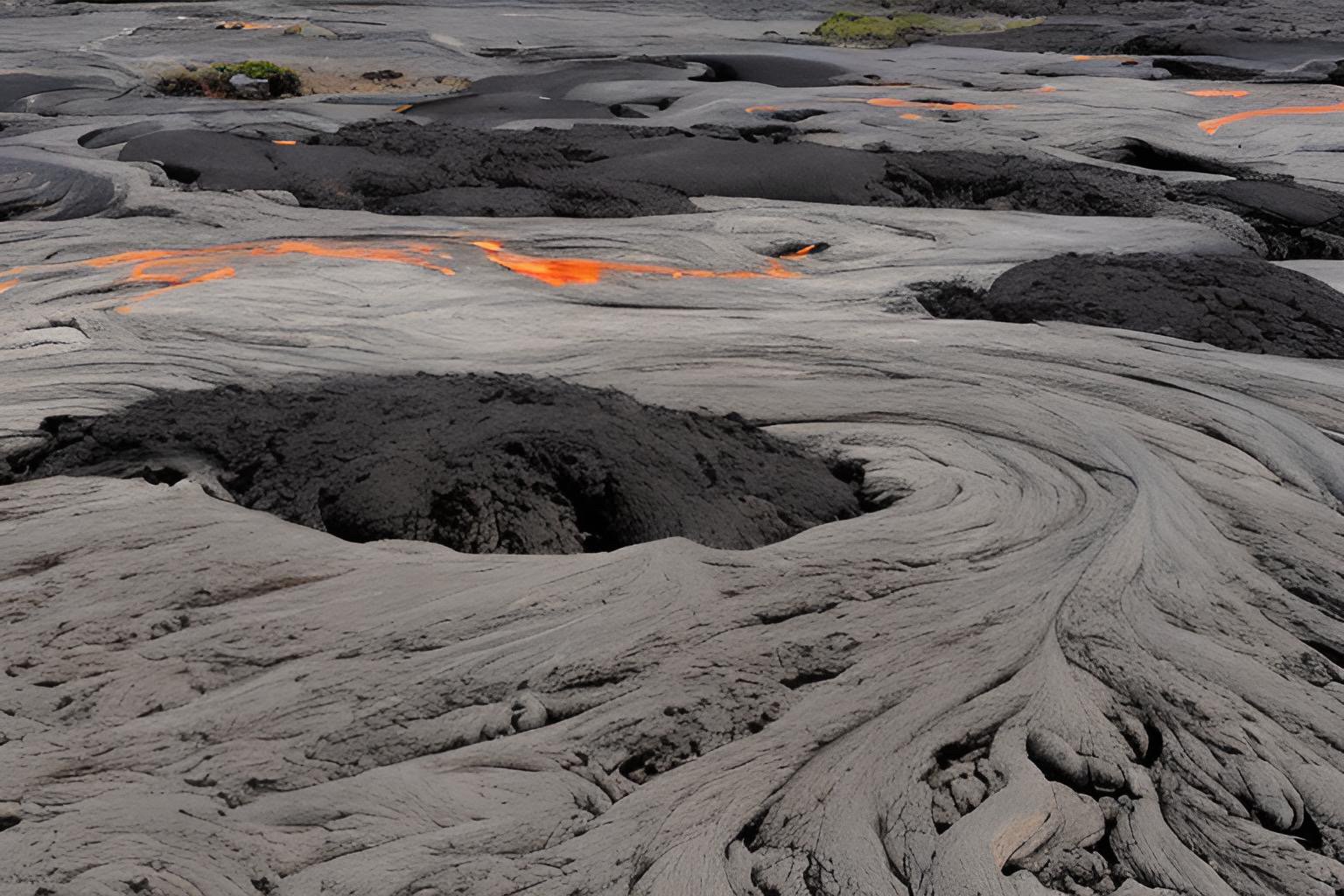
Chapter 4. proto-Earth
1.Hadean proto-Earth
Proto-Earth refers to the very young Earth, before it reached its current size and composition and before the theoretical Giant Impact between the proto-Earth and a Venus size planet named Thiea. We will discuss this more in the Next Chapter. It was a time when our planet was still in the process of forming through the accumulation and collision of various celestial objects like meteorites, comets, asteroids, and planetesimals. We use the term "proto-Earth" to describe the planet around 4.6-4.5 billion years ago during its initial stages of development up to the collision with Theia (Chapter 5).
There are no surviving rocks from this time period, the only objects on earth this old are meteorites that formed along with the formation of the solar system which later impacted Earth. The lack of data makes piecing together the early geologic history of the Earth very challenging. Below we will review what is known and what is still uncertain about the proto-Earth.
-
Description text goes here
-
Description text goes here
-
Item description
2. Heat
-
As a planet increases in size, its likelihood of melting also rises.
In a young, growing planet, there are several sources of heat that contribute to its thermal evolution. These heat sources play a crucial role in shaping the planet's structure, composition, and potential for geological activity. Some of the primary sources of heat in a young, growing planet include:
-
Accretionary heating: As a planet forms through the process of accretion, it accumulates mass by attracting and incorporating surrounding materials, such as dust, gas, and smaller celestial bodies. The kinetic energy of these infalling materials is converted into heat upon impact, which raises the temperature of the growing planet. This process is particularly important during the early stages of planetary formation.
-
Gravitational compression: As a young planet grows in mass, the force of gravity acting on its material increases. This causes the planet to contract and become more compact, which in turn generates heat through the conversion of gravitational potential energy into thermal energy. This process is known as gravitational compression or gravitational heating.
Gravitational Differentiation: As a young planet heats up, it may undergo differentiation, a process in which denser materials, like iron and nickel, sink to the core while lighter materials, like silicates, rise to form the mantle and crust. The movement of materials during differentiation releases gravitational potential energy, which is converted into heat and contributes to the planet's internal temperature.
-
Tidal heating: For planets and moons that are subjected to strong gravitational forces from nearby massive bodies, such as gas giants or binary star systems, tidal heating can be an important source of internal heat. Tidal forces cause the shape of the celestial body to be distorted, generating internal friction and heat. This process is particularly relevant for moons orbiting gas giants, like Jupiter's moon Io, which experiences intense tidal heating due to its proximity to Jupiter and the gravitational interactions with other nearby moons.
-
Radioactive decay: Radioactive isotopes, such as uranium-238, thorium-232, potassium-40, and aluminum-26, are present in varying amounts within a young planet. As these isotopes decay, they release heat, which contributes to the planet's internal temperature. Radioactive decay is an important source of heat in the early solar system, particularly for aluminum-26, which has a relatively short half-life (around 730,000 years) and was more abundant during the early stages of planetary formation.
-
The radioactive isotope of aluminum, specifically aluminum-26 (26Al), played a crucial role as a heat source in the early solar system. Aluminum-26 has a half-life of about 730,000 years, and it decays into magnesium-26 (26Mg) through beta-plus decay, releasing energy in the form of gamma radiation and positrons. This release of energy produces heat that can contribute to the heating of nearby materials, such as dust and rock.
In the early solar system, roughly 4.6 billion years ago, aluminum-26 was more abundant than it is today, likely produced by nucleosynthesis in massive stars and distributed throughout the interstellar medium by supernovae. This isotopic abundance led to a significant amount of heat generation during the formation of planetesimals and planets.
The heat generated by aluminum-26 decay contributed to the melting and differentiation of many early planetesimals and protoplanets. The decay of 26Al provided enough heat to drive the melting of silicate and metallic materials within these bodies, allowing for the separation of denser materials, such as iron, to the core and less dense materials, like silicates, to the mantle and crust.
The presence of aluminum-26 in the early solar system is supported by the study of meteorites, particularly chondrites, which are some of the oldest and most primitive remnants of the solar system's formation. The isotopic composition of magnesium in these meteorites provides evidence for the past presence of aluminum-26, as the 26Mg isotope is a decay product of 26Al.
Overall, the decay of aluminum-26 was a significant heat source in the early solar system, influencing the thermal evolution, melting, and differentiation of planetesimals and protoplanets during their formation.
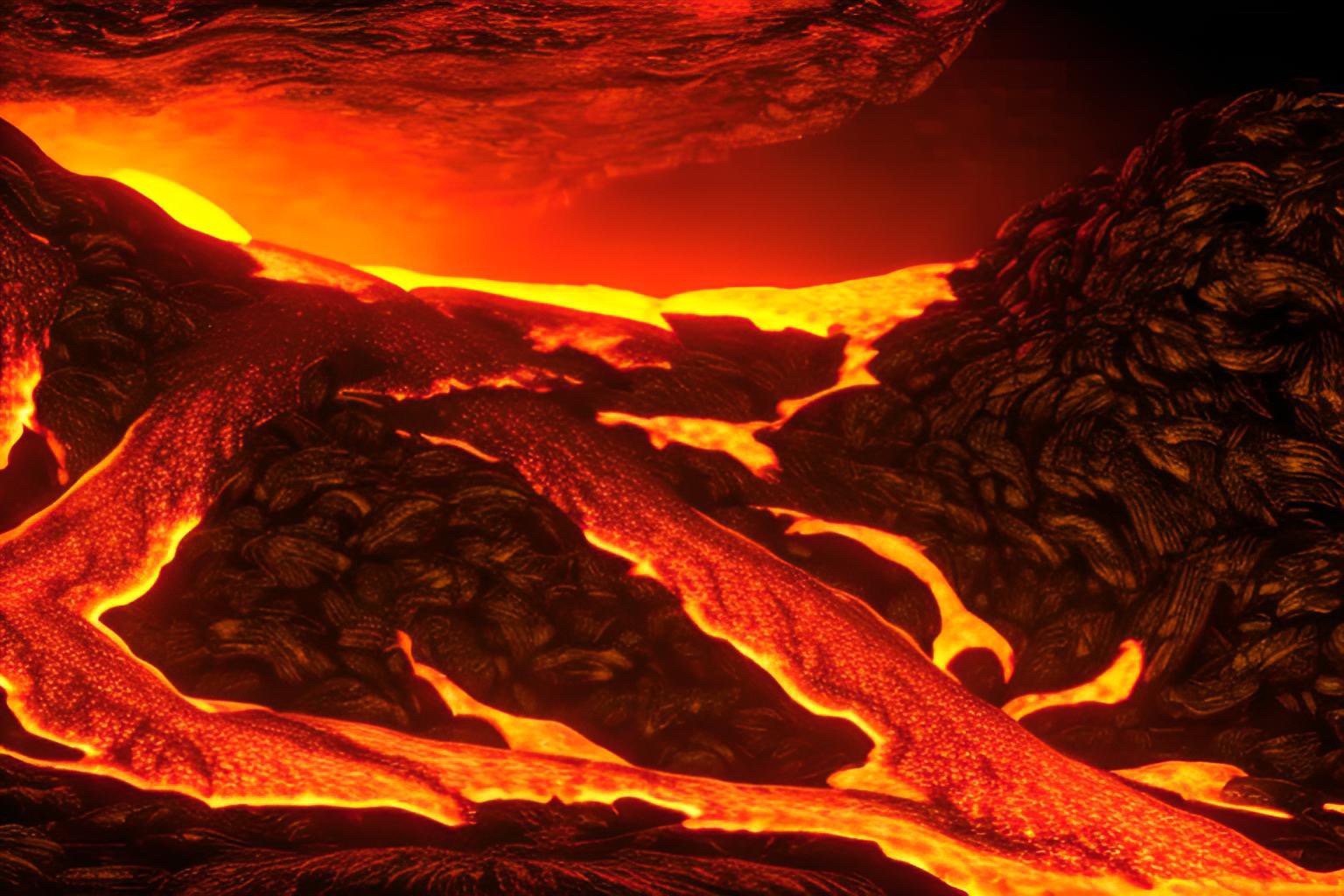
2. Magma Oceans
Magma is molten or partially molten rock, typically located below the surface of a planet. It forms when the temperature and pressure conditions within the Earth's mantle or crust cause rocks to partially or completely melt. Magma consists of a mixture of molten or semi-molten rock, dissolved gases (like water vapor, carbon dioxide, and sulfur dioxide), and sometimes solid mineral crystals.
Magma plays a significant role in the formation of igneous rocks and volcanic activity. When magma rises towards the Earth's surface and cools, it solidifies into igneous rocks. The rate of cooling and the chemical composition of the magma determine the resulting rock's texture and mineral composition. Currently on Earth, most magma is below the Earth’s surface, but as the young planet formed it is likely there were periods of time when the outer layer of the Earth was completely molten.
There are a few known examples of planets with magma oceans, these are located very closer to their suns. The intense heat liquifies the crystalline rock, vaporizing quartz.
-
A magma ocean refers to a large-scale, global layer of molten rock or magma covering a celestial body. It occurs when a significant portion of a planet's or moon's outer layer is heated to such an extent that the solid rocks are melted and form a fluid or semi-fluid state. Magma oceans can be found on newly formed planets, during large-scale impacts, or on planets and moons subjected to intense heating from their host stars or other sources
In young planets, magma oceans can play a significant role in planetary differentiation, a process through which the denser elements (such as iron and nickel) sink towards the core of a planet or moon, while lighter elements (such as silicates) float upwards and form a mantle and crust. This process can lead to the development of distinct layers within the celestial body.
As magma cools and solidifies, gases and other volatiles like water that were previously dissolved in the magma can be released into the atmosphere. This process can contribute to the formation of a planet's or moon's atmosphere and can also lead to the development of volcanic activity.
-
K2-141b is a rocky exoplanet with a radius about 1.5 times that of Earth and a mass around 5 times that of Earth, making it a super-Earth. Its most notable feature is its extremely close orbit to its host star and its ultra-short orbital period. K2-141b orbits its star in just 0.28 Earth days (about 6.7 hours), at a distance much closer than Mercury orbits the Sun.
Due to its proximity to the host star, K2-141b experiences extreme temperatures, with the day side reaching up to 3,000 Kelvin (approximately 2,727°C or 4,940°F). This is hot enough to vaporize rocks, creating a thin atmosphere of vaporized rock including Na, Si and SiO2 and a magma ocean on the day side of the planet. The strong temperature contrast between the day side and the night side causes supersonic winds that can reach speeds of up to several kilometers per second, carrying the vaporized rock particles to the cooler night side, where they can condense and precipitate as rocky rain.
K2-141b is tidally locked to its star, meaning that the same side of the planet always faces the star, much like how the same side of the Moon always faces Earth. This results in a perpetual day side and night side, with the day side experiencing scorching temperatures and the night side being significantly cooler.
-
Io, one of Jupiter's largest moons, is not considered to be entirely molten, but it does have extensive volcanic activity and a partially molten interior. It is the most volcanically active body in our solar system, with over 400 active volcanoes. This intense volcanic activity is primarily driven by tidal heating, which is caused by the gravitational interactions between Io, Jupiter, and its neighboring moons, Europa and Ganymede.
As Io orbits Jupiter, it experiences strong tidal forces that cause its shape to be constantly distorted. This deformation generates friction and heat within Io's interior, which in turn melts parts of its mantle, leading to the creation of magma. The magma rises to the surface through volcanic eruptions, creating vast lava fields and volcanic plumes that can reach hundreds of kilometers in height.
Although Io's interior is not completely molten, it is believed to have a partially molten asthenosphere (a layer of the mantle below the lithosphere) composed of silicate rock and possibly a molten or partially molten core made of iron and sulfur. The intense tidal heating and continuous volcanic activity prevent Io from cooling down and forming a thick, stable crust like that found on other celestial bodies, such as Earth and the Moon. Instead, Io has a thin, young crust primarily composed of sulfur and silicate compounds, which is constantly being resurfaced by volcanic eruptions and lava flows.
-
Item description
3.Magma Composition
Modern igneous rocks and the magma they come from are categorized by their silica (SiO2) content and fall in one of three categories:
-
This type of magma has the lowest silica content (45-55%) and is rich in iron, magnesium, and calcium. It is generally more fluid and less viscous than other types of magma. Basaltic magma typically forms at mid-ocean ridges and hotspots, creating basaltic rocks like basalt and gabbro.
-
Andesitic magma has an intermediate silica content (55-65%) and contains a mix of minerals, including plagioclase feldspar, amphibole, and pyroxene. It is more viscous than basaltic magma and forms in subduction zones where the oceanic crust sinks beneath the continental crust. The resulting rocks are typically andesite and diorite.
-
Rhyolitic magma has the highest silica content (65-75%) and is rich in potassium and sodium. It is the most viscous of the three magma types, which can lead to explosive volcanic eruptions when gases are trapped within the magma. Rhyolitic magma is typically found in continental crust settings and forms rocks like rhyolite and granite.
No rocks have been preserved from the earliest period of Earth’s history.
But it is believed that modern magmas are evolved/enriched/separated magmas from a theoretical starting magma that closely represented the original composition of Earth.
Questions:
Models of large planetary collisions result in high enough energy to melt all or most of a planet. The melting alone would cause some mixing, but the shaking action of the resulting earthquakes would be good at sorting material???
Silicates are the cornerstone of Earth's geology, shaping our planet's crust and defining its unique composition, while driving the processes that have sculpted its dynamic landscapes over billions of years.
3.Sillicates
Silicates are a class of minerals that are composed primarily of silicon and oxygen, with the basic chemical formula of SiO4. These minerals make up the majority of Earth's crust, and are the most abundant type of mineral found on the planet. Silicates can be divided into various groups based on their structure. Before we explore the tetrahedral structure of SiO4 let’s go over some silicon based vocabulary:
-
Silicon is a chemical element with the symbol Si and atomic number 14. It is a metalloid, which means it has properties between those of metals and nonmetals. Silicon is the second most abundant element in Earth's crust, after oxygen. It is commonly found in the form of silicon dioxide (silica) and silicate minerals. Silicon is widely used in the electronics industry for semiconductors, as well as in the production of solar panels, glass, and various alloys.
-
Silica, also known as silicon dioxide (SiO2), is a chemical compound consisting of one silicon atom and two oxygen atoms. Silica occurs in both crystalline and amorphous forms, with quartz being a well-known example of the crystalline form. Silica is the primary component of sand and is used as a raw material in the production of glass, ceramics, and concrete. In nature, silica is commonly found in rocks, soils, and as a constituent of the Earth's crust.
-
Silicates are a group of minerals composed of silicon, oxygen, and one or more additional elements, such as aluminum, magnesium, iron, calcium, sodium, and potassium. The basic structural unit of silicate minerals is the silica tetrahedron (SiO4), in which a silicon atom is surrounded by four oxygen atoms. Silicate minerals can be classified into various groups based on the arrangement of these tetrahedra, such as nesosilicates, sorosilicates, cyclosilicates, inosilicates, phyllosilicates, and tectosilicates. Silicates make up the majority of Earth's crust and mantle and are crucial components of many rock types, such as granite, basalt, and gneiss.
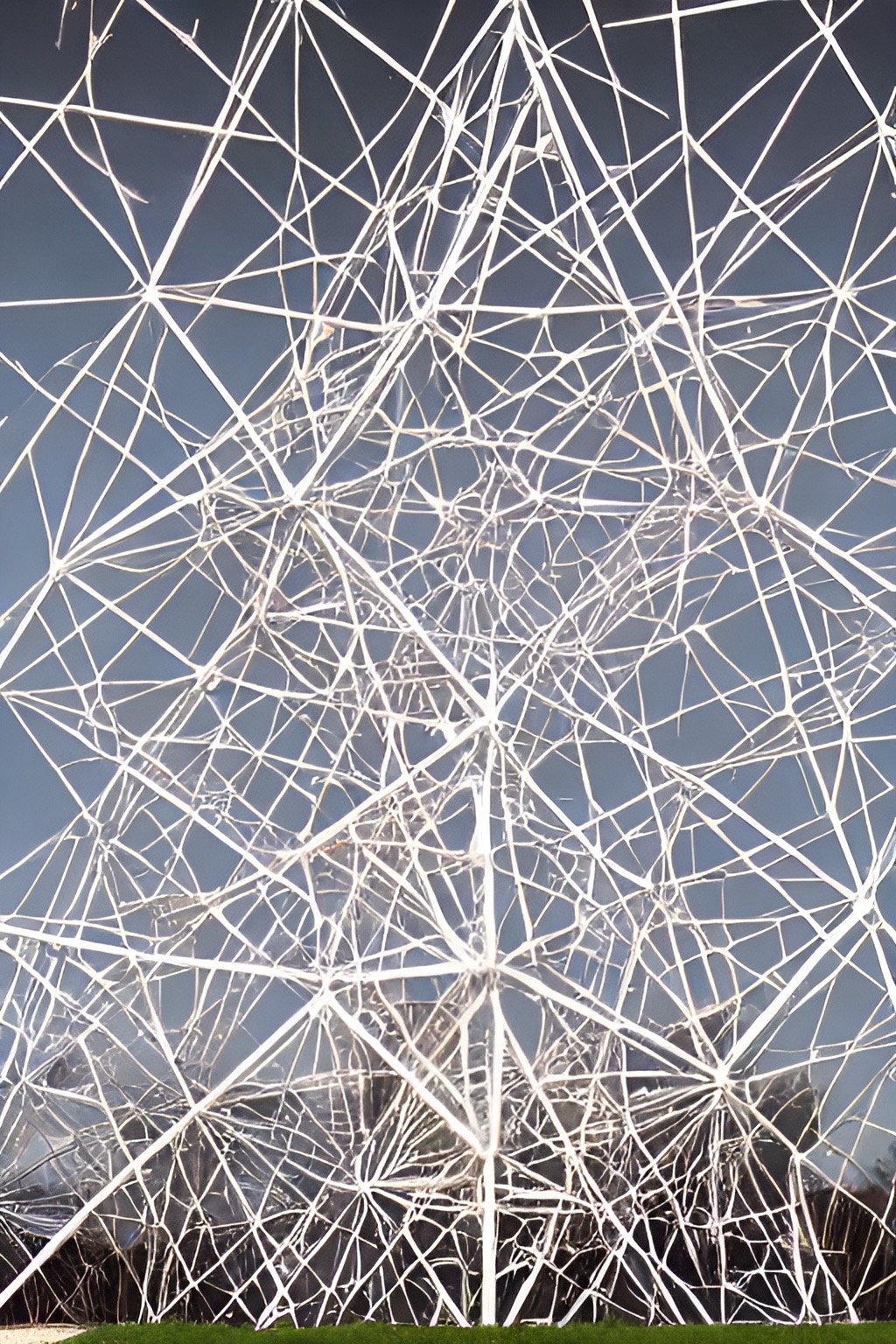
The Si-O Bond:
The electronegativity difference between oxygen and silicon in a Si-O bond within a silicate tetrahedron is 1.54, as calculated using the Pauling electronegativity scale. This value indicates that the Si-O bond has a polar covalent character, meaning that the electrons are shared unequally between the two atoms. In this bond, the oxygen atom attracts the electrons more strongly than the silicon atom, leading to an uneven distribution of electron density and the resulting polarity.
4.Tetrahedrals
The silicate tetrahedron consists of one silicon atom (Si) at the center and four oxygen atoms (O) surrounding it at the corners of a tetrahedron. The silicon atom forms covalent bonds with the oxygen atoms, resulting in the SiO4 unit with a net charge of -4.
This SiO4 tetrahedron is the basic building block of silicate minerals, which are the most abundant group of minerals in the Earth's crust. The tetrahedra can combine in various ways to form a wide range of silicate minerals with different structures and properties.
-
Nesosilicates (isolated tetrahedra): The tetrahedra do not share any oxygen atoms with each other and are bonded to other cations (such as magnesium or iron) to balance the charge. Examples include olivine and garnet.
-
Sorosilicates (double tetrahedra): Two silicate tetrahedra share one oxygen atom, creating an Si2O7 unit with a net charge of -6. An example is the mineral epidote.
-
Inosilicates (single and double chains): The tetrahedra can link together to form single or double chains by sharing two oxygen atoms, respectively. Examples include pyroxene and diopside.
-
Ring silicates, also known as cyclosilicates, are a subclass of silicate minerals characterized by silicate tetrahedra (SiO4) that are arranged in rings. In these structures, the SiO4 tetrahedra share two oxygen atoms with neighboring tetrahedra, forming closed ring-like structures. The rings can have different sizes, with the most common ones consisting of 3, 4, or 6 tetrahedra. These rings are connected to other cations or complex groups, which provide charge balance and help define the overall structure of the mineral.
-
Double-chain silicates link together by two or three tetrahedra sharing oxygens. Examples include amphibole and tremolite.
-
Phyllosilicates (sheet silicates): The tetrahedra share three oxygen atoms and form continuous two-dimensional sheets. Examples include micas and clay minerals.
-
Tectosilicates (framework silicates): All four oxygen atoms in the tetrahedra are shared with neighboring tetrahedra, creating a three-dimensional framework structure. Examples include quartz and feldspars.
Shared Oxygens
Tetrahedral Structures
Formation Process - Texture
-
Extrusive (or volcanic) igneous rocks: These rocks form when magma reaches the Earth's surface through volcanic eruptions and cools rapidly upon contact with the air or water. The rapid cooling usually results in fine-grained or glassy textures. Examples include basalt, andesite, and rhyolite.
-
Intrusive (or plutonic) igneous rocks: These rocks form when magma cools and solidifies below the Earth's surface, resulting in a slow cooling process. The slow cooling allows crystals to grow larger, creating coarse-grained or phaneritic textures. Examples include granite, diorite, and gabbro.