Earth Unveiled: A Comprehensive 3D VR Model of the Earth's Interior
"Earth Unveiled" is an ambitious initiative aimed at creating an immersive, interactive 3D VR model of Earth's interior. Our mission is to bring the world's latest geological and geophysical research to life, making it accessible and engaging to both scientists and the general public.
This project is a collaborative effort, inviting researchers globally to contribute their findings and data to enrich the depth of our model. Our model will not only portray current understanding of Earth's structure but will also highlight areas of persistent uncertainty, emphasizing the necessity of continuous exploration and study.
In blending VR technology with cutting-edge earth science, we aim to foster curiosity about the planet's hidden layers and inspire future research. "Earth Unveiled" is more than a project; it's a journey into the heart of our shared home, designed to deepen our collective understanding of the Earth.
Building Earth in Virtual Reality
Building Earth in Virtual Reality
Using Blender to merge NASA 3D Earth data with Dr Sanne Cottaar's Large Low Shear Velocity Province (LLSVP) Blobs
Wrapping VR Earth with maps built in ArcGIS Pro
Mapping the cratons, the oldest parts of each continent.
Earth in 3D VR
Plate Tectonics Earth
Mapping hot spots and spreading ridges.
Earth's Engine - Look Under the Hood
Primary Geologic Features Included Within Earth Unveiled
-
The Earth's crust is the outermost shell of our planet, forming the surface where we live and interact. It's made up of a variety of rocks and minerals and is fundamentally split into two types: oceanic crust and continental crust.
The continental crust underlies the landmasses of the Earth. It is generally thicker, ranging from about 30 to 70 kilometers (average around 40 km), and comprises lighter, silica-rich rocks like granite. This type of crust is less dense and more buoyant, causing it to "float" higher on the semi-fluid asthenosphere below. It's also significantly older, with some parts dating back billions of years.
On the other hand, the oceanic crust underlies the ocean basins and is considerably thinner, usually about 5 to 10 kilometers thick. It consists mainly of denser, silica-poor rocks such as basalt, which contain more iron and magnesium. The oceanic crust is younger, as it is continuously being created at mid-ocean ridges and destroyed at subduction zones.
The Earth's crust is not a solid shell but is broken into several large pieces known as tectonic plates. These plates float atop the semi-fluid asthenosphere and their movements are responsible for earthquakes, volcanic activity, and the creation of mountain ranges.
Although it is the thinnest layer of the Earth, the crust is vital to our existence. It's where all life as we know it resides, and it hosts essential resources like water, minerals, and fossil fuels that sustain our societies.
Composition:
Continental Crust: It is predominantly composed of lighter, silica-rich rocks such as granite. These rocks are less dense, due to their higher content of elements like silicon and oxygen.
Oceanic Crust: It is mainly composed of denser, silica-poor rocks like basalt. These rocks have a higher concentration of heavier elements like magnesium and iron.
Thickness:
Continental Crust: It is considerably thicker, ranging from about 30 to 70 kilometers (average about 40 km). The thickness varies significantly, with mountainous regions having the thickest crust.
Oceanic Crust: It is much thinner, usually about 5 to 10 kilometers thick.
Density:
Continental Crust: Less dense, with an average density of about 2.7 grams per cubic centimeter.
Oceanic Crust: More dense, with an average density of about 3.0 grams per cubic centimeter.
Age:
Continental Crust: It is generally much older, with some areas dating back several billion years.
Oceanic Crust: It is considerably younger, as it's constantly being created at mid-ocean ridges and destroyed at subduction zones. The oldest oceanic crust is "only" about 200 million years old.
Buoyancy:
Continental Crust: Due to its lower density, continental crust is more buoyant and "floats" higher on the semi-fluid asthenosphere beneath the lithosphere.
Oceanic Crust: Being denser, oceanic crust is less buoyant and "sinks" lower into the asthenosphere.
These differences lead to significant implications for the geological activity observed at the Earth's surface. For example, when oceanic and continental crust collide, the denser oceanic crust tends to be forced, or subducted, under the lighter continental crust, leading to the formation of mountain ranges and volcanic activity.
-
(Seismic Velocity data & Gravity Data)
In the context of plate tectonics, "downgoing slabs" or "subducting slabs" refer to sections of the Earth's lithosphere (the rigid outer layer composed of the crust and uppermost mantle) that are sinking into the mantle. This process is a key component of subduction zones, where one tectonic plate converges with another and is forced downwards.
The driving force behind this process is the relative density of the tectonic plates. Oceanic crust is denser than continental crust, largely due to its composition of basaltic rocks. As a result, when an oceanic plate converges with a less dense continental plate, the denser oceanic plate is forced underneath, creating a subduction zone. This process can also occur between two oceanic plates, with the older (and therefore denser) plate typically being the one that subducts.
As the downgoing slab sinks into the mantle, it also carries water and other volatiles into the mantle. The introduction of these substances can lower the melting point of the mantle material, leading to the formation of magma. This magma can rise through the crust to form volcanic arcs, which are commonly seen at subduction zones.
Over millions of years, these subducted slabs can reach great depths in the mantle, and their sinking can drive mantle convection, a process that is a primary driving force of plate tectonics.
There is also interest in "buried" or "stagnant" slabs, which are slabs that have become "stuck" at certain depths within the mantle, often at the boundary between the upper and lower mantle (around 660 kilometers depth). These stagnant slabs can have significant impacts on mantle convection and the movement of overlying tectonic plates. The mechanisms behind slab stagnation are not fully understood and are a subject of ongoing research in geophysics and tectonics.
-
Spreading ridges, also known as mid-ocean ridges, are significant geological features that occur at the boundaries between tectonic plates where new oceanic crust is being formed. This process is known as seafloor spreading, and it's a fundamental aspect of plate tectonics.
Formation and Structure: As molten rock (magma) rises from the mantle to the crust, it cools and solidifies to form new crust. This process takes place along the length of the spreading ridge, which can extend for thousands of kilometers across an ocean basin. The continuous formation of new crust pushes the older crust away from the ridge, causing the tectonic plates on either side to move apart in a process called divergence.
Physical Characteristics: Spreading ridges are characterized by a central rift valley, flanked by parallel mountain ranges or "ridges". The central rift is where the magma reaches the seafloor and new crust is formed. These features are generally broad and rise 2-3 km above the seafloor, with the summit of the ridge often lying a few kilometers below the ocean surface.
Biological Significance: The volcanic activity associated with spreading ridges brings heat and nutrients to the otherwise cold and nutrient-poor deep ocean environment. This can create deep-sea hydrothermal vents, which support diverse and unique ecosystems that can survive in the absence of sunlight.
Geological Significance: The process of seafloor spreading at mid-ocean ridges provides crucial evidence for the theory of plate tectonics. The age of the oceanic crust increases with distance from the spreading ridge, a pattern that is consistent with the continuous formation and outward movement of the crust from these locations. Furthermore, patterns of magnetic reversals recorded in the oceanic crust provide additional supporting evidence.
Examples: The Mid-Atlantic Ridge, which splits the Atlantic Ocean between the Americas on the west and Europe and Africa on the east, and the East Pacific Rise in the Pacific Ocean, are two of the most well-known examples of spreading ridges.
-
The Mohorovičić Discontinuity, often referred to as the Moho, is the boundary separating the Earth's crust from the underlying mantle. It's named after the Croatian seismologist Andrija Mohorovičić, who discovered it in 1909.
Mohorovičić made this discovery while studying seismic waves generated by earthquakes. He noticed that the velocity of seismic waves increases dramatically at a certain depth below the Earth's surface. This sudden increase in velocity, which is due to a change in the composition and density of the rocks, marks the boundary between the crust and the mantle.
The depth of the Moho varies significantly across the Earth. Under the oceanic crust, it's usually found at depths of about 5 to 10 kilometers. However, beneath the continental crust, it can be much deeper - often around 30 to 50 kilometers, but can go up to 70 kilometers under certain mountain ranges.
The Moho is an important concept in geology and geophysics, as it provides a clear demarcation between the Earth's outermost layer (the crust) and the vast mantle beneath. Despite its importance, it remains one of the least explored parts of the Earth, primarily due to the technical challenges and high costs associated with drilling to such depths.
-
The asthenosphere is a region of the Earth's upper mantle that lies beneath the lithosphere and above the lower mantle. The depth of the asthenosphere varies, but it generally extends from about 100 kilometers (62 miles) to about 410 kilometers (255 miles) beneath the Earth's surface.
Composition: Like the rest of the mantle, the asthenosphere is composed primarily of silicate rocks rich in iron and magnesium. The dominant rock in this layer is peridotite.
Physical State: While it's still a solid, the asthenosphere is highly viscous and partially molten, meaning it can flow and deform slowly over time. This is due to the high temperatures and pressures at these depths, which can cause small amounts of partial melting within the rock.
Role in Plate Tectonics: The asthenosphere is crucial to the movement of tectonic plates. The lithospheric plates "float" on the semi-fluid asthenosphere, and its convective motion helps drive the movement of these plates. This process can lead to the creation of mountains, earthquakes, and volcanic activity at the Earth's surface.
Temperature and Pressure: The temperatures in the asthenosphere range from about 1300 to 2200 degrees Celsius (2372 to 3992 degrees Fahrenheit). The pressure, like temperature, also increases with depth.
The asthenosphere is a key part of the Earth's structure, and understanding its properties and behavior is crucial to our knowledge of plate tectonics and many geological processes.
-
Between 410 and 660 kilometers depth in the Earth's mantle, there is a region known as the transition zone. This zone is characterized by significant changes in mineral structures due to the increasing pressure and temperature conditions. Here, two main phase transitions are known to occur, affecting the mineral olivine, which makes up a large portion of the mantle's composition. These transformations result in an increase in density and a change in the arrangement of atoms within the mineral structure.
At ~410 km depth: Olivine, in its α-phase (alpha-phase, also known as forsterite or α-olivine), changes to a high-pressure polymorph called wadsleyite or β-phase (beta-phase, also known as beta-spinel). This transition is primarily pressure-induced and causes a significant increase in density. The structural change involves the rearrangement of the silicon-oxygen tetrahedra that make up the basic structure of these minerals.
At ~520 km depth: Wadsleyite transitions to another high-pressure form of olivine, known as ringwoodite, which remains stable until a depth of about 660 km. Ringwoodite has a spinel structure where each silicon atom is surrounded by four oxygen atoms arranged at the corners of a regular tetrahedron.
At ~660 km depth: Ringwoodite decomposes into two new minerals - silicate perovskite (which eventually becomes the dominant mineral in the lower mantle) and ferropericlase. This phase transition is considered one of the most important in terms of mantle dynamics, as it marks the boundary between the transition zone and the lower mantle. This boundary is sometimes referred to as the "660-kilometer discontinuity."
These phase transitions are detected through the study of seismic waves, which change speed when they encounter different densities and elastic properties of materials. The transitions also have significant effects on the behavior of the mantle and the dynamics of the Earth's interior, including the movement of tectonic plates and the Earth's heat budget.
-
The Gutenberg Discontinuity, also known as the core-mantle boundary (CMB), is the boundary that separates the Earth's mantle from its core. It's located approximately 2,890 kilometers (1,800 miles) beneath the Earth's surface. The discontinuity is named after Beno Gutenberg, a seismologist who made several important contributions to the study of the Earth's interior.
One of the significant characteristics of the Gutenberg Discontinuity is a sharp increase in density. The mantle is composed mainly of silicate rocks, while the core is made primarily of iron and nickel, which are much denser.
Seismic waves also behave differently when they encounter this boundary, which is how it was first discovered. P-waves (primary waves, which are compressional) slow down dramatically as they enter the outer core because it is a liquid, and S-waves (secondary waves, which are shear waves) cannot travel through the outer core at all due to its liquid state. This leads to a "shadow zone" for S-waves on the opposite side of the Earth from a seismic event.
This boundary represents not just a physical and compositional boundary, but also a thermal one. The core is much hotter than the mantle, and this heat drives powerful processes, such as the creation of Earth's magnetic field and mantle convection, which is a driving force behind plate tectonics.
The exact nature of the Gutenberg Discontinuity and the core-mantle boundary region is still a subject of ongoing scientific study. For instance, some research suggests that there may be a "D'' layer (a region several hundred kilometers thick just above the CMB) with complex behaviors that could influence both core and mantle dynamics.
-
Completion of Earth Unveiled will mark the opportunity to go back in time and see how Earth has evolved from it’s very beginning.
The first step will be mapping paleo reconstruction data to assemble the first known supercontinent Rodinia.
Rodinia is the name given to a supercontinent that geologists believe existed between approximately 1.3 billion and 900 million years ago during the Neoproterozoic era. Its name comes from the Russian word "родить" (rodit'), which means "to give birth" – a fitting name, considering that the breakup of Rodinia gave rise to much of the continental configuration we see today.
Formation and Composition: Rodinia formed through the process of plate tectonics, as smaller continental landmasses collided and fused together. It is thought to have been composed of most or all of Earth's continental crust at the time, although the exact configuration of the continents within Rodinia is still a matter of scientific debate.
Breakup: The breakup of Rodinia is believed to have begun around 800 to 750 million years ago. This event is associated with significant geological activity, including the creation of rifts and the eruption of large volumes of volcanic rock. The breakup of Rodinia eventually led to the formation of a new ocean called the Iapetus Ocean, and later, a new supercontinent called Pangaea.
Significance: The formation and breakup of Rodinia had profound impacts on Earth's climate, life, and the geochemical cycles. The breakup, for example, is thought to have led to a series of global glaciations, known as the Snowball Earth events. These extreme ice ages could have played a significant role in the evolution of multicellular life, which appeared shortly after Rodinia's breakup.
Evidence: Evidence for Rodinia's existence comes from a range of geological sources, including the distribution of certain rock types, paleomagnetic data, and the geological history of existing continents. However, because the supercontinent existed so long ago, many details about its history and configuration remain uncertain.
“What processes shaped Earth into its current state?”
Follow along as we explore:
The Story of Earth
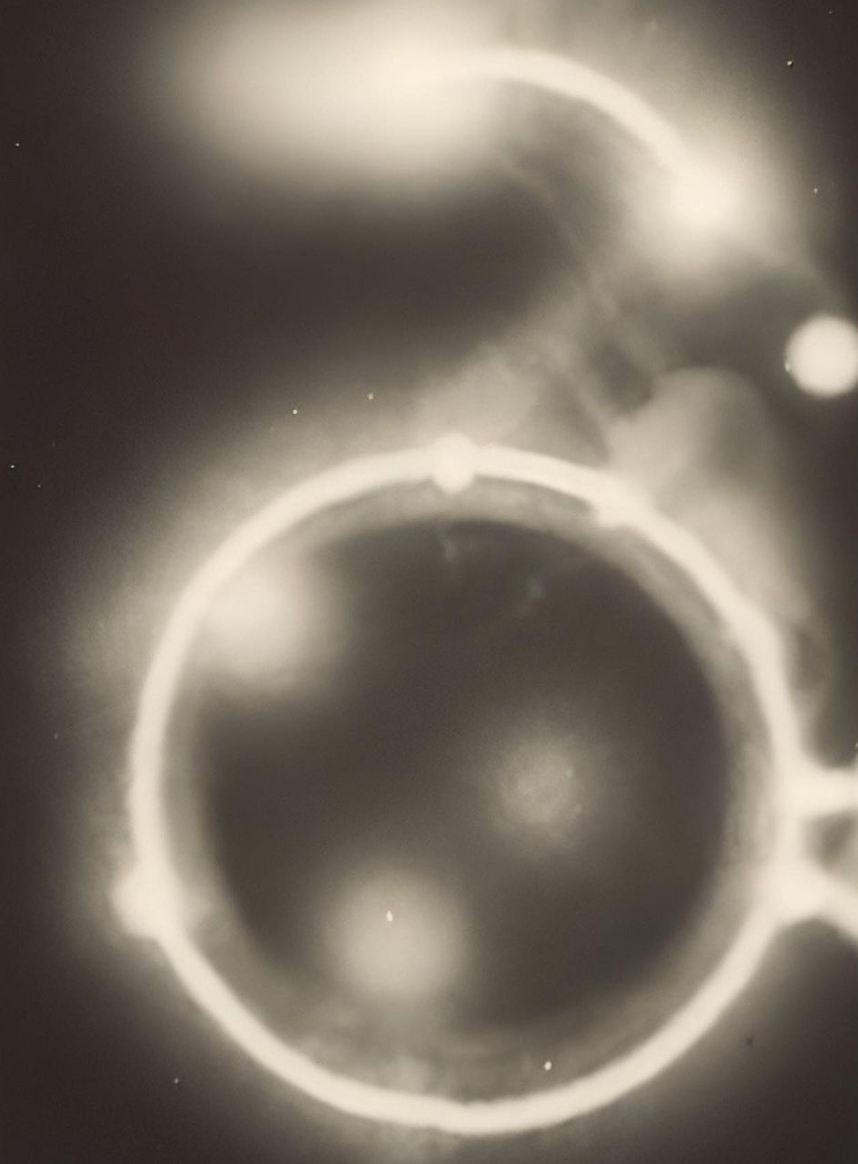
intermission
Part 2: Draft Timeline